Катализаторы на основе платины на различных углеродных носителях и проводящих полимерах для применения в топливных элементах с прямым метанолом:обзор
Аннотация
Металлы в виде наночастиц на основе платины (Pt) привлекли большое внимание и являются наиболее популярными катализаторами для топливных элементов с прямым метанолом (DMFC). Однако высокая стоимость Pt-катализаторов, медленное кинетическое окисление и образование промежуточных молекул CO во время реакции окисления метанола (MOR) являются основными проблемами, связанными с монометаллическими Pt-катализаторами. Недавние исследования сосредоточены на использовании либо сплавов Pt, таких как металлы Fe, Ni, Co, Rh, Ru, Co и Sn, либо материалов углеродного носителя для улучшения каталитических характеристик Pt. В последние годы катализаторы из Pt и Pt сплавов, поддерживаемые большим потенциалом углеродных материалов, таких как MWCNT, CNF, CNT, CNC, CMS, CNT, CB и графен, вызвали значительный интерес из-за их значительных свойств, которые могут способствовать отличному MOR. и производительность DMFC. В этой обзорной статье резюмируется разработка вышеупомянутых сплавов и вспомогательных материалов, связанных с уменьшением использования Pt, улучшением стабильности и улучшенными электрокаталитическими характеристиками Pt в DMFC. Наконец, представлено обсуждение каждого катализатора и носителя с точки зрения морфологии, электрокаталитической активности, структурных характеристик и характеристик топливных элементов.
Введение
Технология топливных элементов получила широкое внимание во всем мире. Топливные элементы (ТЭ) представляют собой перспективную альтернативную технологию производства электроэнергии, которая преобразует химическую энергию в электрическую посредством электрохимической реакции [1, 2]. Более того, в технологии топливных элементов основной упор в технологии топливных элементов делается на создание недорогой продукции, что позволяет добиться высокой производительности системы топливных элементов и открыть для себя прочные материалы. Тем не менее, общие проблемы, которые возникают в современной технологии топливных элементов, состоят в том, что системы связаны с высокими внутренними затратами и низкой долговечностью [1]. Несмотря на перспективность использования в качестве топливного элемента, топливные элементы с прямым метанолом (DMFC) имеют проблемы и ограничения, что побуждает исследователей изучать методы повышения эффективности и производительности DMFC. Многие проблемы с DMFC были выявлены и остаются нерешенными, в том числе переход метанольного топлива с анодного электрода на катодный электрод [3,4,5]. Плохая производительность, вызванная низкой скоростью кинетики, нестабильностью катализатора, а также регулированием температуры и воды. [6,7,8].
В последнее время было проведено множество исследований топливных элементов, включая DMFC, топливный элемент с протонообменной мембраной (PEMFC), твердооксидный топливный элемент (SOFC) и т. Д., Которые являются популярными технологиями топливных элементов. В качестве нового источника энергии DMFC могут использоваться для мобильных и стационарных приложений [9, 10]. Многие исследования были достигнуты в области топливных элементов. Среди топливных элементов DMFC широко изучались в последние годы [11,12,13,14,15,16] из-за их множества преимуществ, таких как высокая удельная мощность, простота обращения с топливом, легкость зарядки и низкий уровень воздействия на окружающую среду. удар [17, 18]. Однако несколько технических проблем для коммерциализации DMFC остаются нерешенными, включая переход метанола, низкие скорости химических реакций и отравление катализатора. Однако DMFC по-прежнему привлекают внимание многих исследователей и стали наиболее популярными топливными элементами из-за их работы при низких температурах (системы DMFC работают при 373 K). Благодаря преимуществам DMFC, заключающимся в высокой энергоэффективности и быстром запуске системы, технология DMFC очень подходит для использования в качестве источников питания в жилых помещениях, аккумуляторов в мобильных устройствах и в качестве автомобильного топлива [19,20,21,22]. Кроме того, концепция DMFC может быть дополнительно изучена для поиска альтернативных источников топлива, таких как природный газ и биомасса, а также ферментация сельскохозяйственных продуктов для производства этанола, чтобы минимизировать зависимость от небезопасных источников энергии [14].
В DMFC на анодную сторону подается раствор метанола, который подвергается электроокислению до диоксида углерода (CO 2 ) через реакцию ниже:
$$ {\ mathrm {CH}} _ 3 \ mathrm {OH} + {\ mathrm {H}} _ 2 \ to {\ mathrm {CO}} _ 2 + 6 {\ mathrm {H}} ^ {+} + 6 { \ mathrm {e}} ^ {\ hbox {-}} $$ (1)В то время как на катодной стороне протон, кислород (из воздуха) восстанавливается до воды:
$$ 3/2 \ {\ mathrm {O}} _ 2 + 6 {\ mathrm {H}} ^ {+} + 6 \ {\ mathrm {e}} ^ {\ hbox {-}} \ to 3 {\ mathrm {H}} _ 2 \ mathrm {O} $$ (2)Чистое уравнение реакции DMFC можно резюмировать следующим образом:
$$ {\ mathrm {CH}} _ 3 \ mathrm {OH} +3/2 {\ mathrm {O}} _ 2 \ to {\ mathrm {CO}} _ 2 + 2 {\ mathrm {H}} _ 2 \ mathrm { O} $$ (3)В системах DMFC существует два типа режимов DMFC:активный и пассивный режимы [23,24,25]. В активной системе DMFC выходной поток пакета DMFC рециркулируется посредством регулирования подачи жидкого метанола с обратной связью. Между тем, жидкий метанол в потоке анода контролируется датчиком концентрации метанола, который играет важную роль в обеспечении достаточного впрыска дополнительного количества метанола и воды для восстановления этого топлива на основе заданной концентрации. Существует несколько типов датчиков концентрации метанола, которые используются в системе DMFC для контроля и поддержания исходной концентрации метанола [17]. Обычно жидкий метанол подается на сторону анода с помощью перистальтического насоса, в то время как окружающий воздух, содержащий кислород, подается на сторону катода с помощью воздуходувки или вентилятора [16]. В пассивном режиме системы DMFC жидкий метанол непрерывно подается в систему. Эта пассивная концепция очень привлекательна для системы DMFC [26,27,28]. Понятие пассивного означает, что система работает полностью автономно без каких-либо вспомогательных устройств. Концепция пассивного DMFC означает, что система работает полностью автономно без какой-либо помощи внешнего устройства для перекачивания метанола и нагнетания воздуха в дымовую трубу. В пассивном режиме системы DMFC слой катализатора будет снабжаться метанолом и кислородом в качестве реагентов. Во время реакции окисления метанола (MOR) CO 2 и вода будет удаляться из клетки пассивными способами, то есть диффузией, естественной конвекцией, капиллярным действием и т. д. [20]. DMFC в пассивном режиме кажется более выгодным по сравнению с DMFC в активном режиме с точки зрения более простой, компактной конструкции и низкой стоимости. Сложная конструкция системы и элементов управления может быть недостатком активного режима DMFC [21]. С точки зрения практического использования активный режим DMFC кажется более подходящим для мощных систем, тогда как пассивный режим DMFC больше подходит для использования в условиях низкого энергопотребления [22].
На рисунке 1 показана установка и конструкция однокамерного DMFC. Одноэлементный пакет DMFC состоит из пятислойной мембранной электродной сборки (MEA), зажатой между двумя пластинами, которые являются анодом и катодом. Со стороны анода жидкий метанол (содержащий метанол и деионизированную воду) и абсолютный метанол втекают в канал с помощью перистальтического насоса. Со стороны катода воздух закачивается в топливный элемент с помощью ротаметра. Контроллер температуры в стеке DMFC используется для поддержания рабочей температуры в ячейке с помощью дополнительного нагревательного устройства. Электронное нагрузочное устройство используется для изменения плотности тока на разные уровни и измерения соответствующих значений напряжения. Производительность ячейки контролируется электрохимической рабочей станцией, а производство CO 2 как конечный продукт общей реакции измеряется CO 2 детектор концентрации [23]. В топливных элементах с прямым метанолом существует несколько важных рабочих параметров, которые необходимо учитывать во время экспериментального исследования, а именно:(i) рабочая температура, (ii) концентрация метанола и (iii) входные скорости потока исходного раствора метанола и воздуха [23]. . На рис. 2a, b показаны активный и пассивный режим DMFC соответственно.
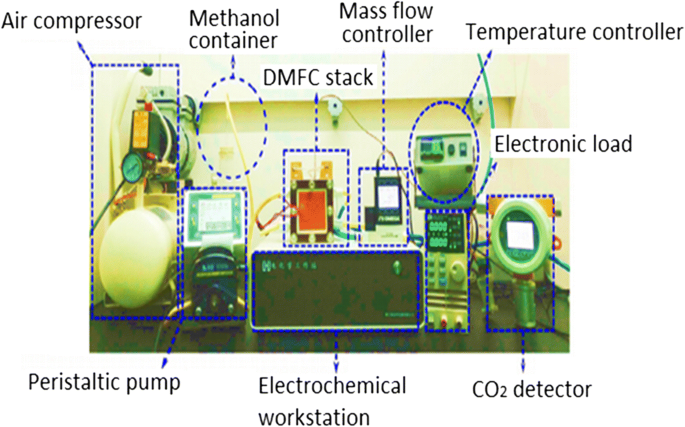
Общая экспериментальная установка для DMFC с одной ячейкой [23]
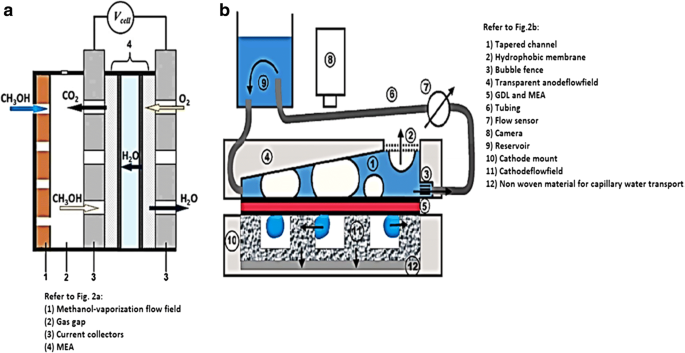
Принципиальная схема а активный режим [24] и b пассивный режим [25] DMFC
Этот обзор будет посвящен недавнему прогрессу в исследованиях и разработках носителя катализатора на основе катализатора Pt в качестве благородного катализатора в DMFC. Мы включаем активность катализаторов на основе Pt в сочетании со сплавами, металлами, переходными металлами, карбидами металлов, нитридами металлов и различными углеродистыми частицами, такими как графен / оксид графена (G / GO), углеродные нанотрубки (CNT), углеродные нановолокна ( CNF), углеродная нанопленка (CNC), углеродная сажа (CB), многослойные углеродные нанотрубки (MWCNT) и углеродные мезопористые (CMS) носители, а также проводящие полимеры, такие как полианилин (PANi) и полипиррол (Ppy) в качестве носителя. материал. Для приготовления катализаторов на основе Pt можно применять многие методы синтеза. Наиболее распространенными методами, применяемыми для получения наноразмерных частиц Pt, являются пропитка [29,30,31,32,33,34], гидротермальные методы [35,36,37,38,39,40,41], микроэмульсия [42,43, 44,45] и редукции [46, 47]. Как правило, способ приготовления может влиять на морфологию и размер частиц катализатора; таким образом, выбор метода синтеза катализатора очень важен.
Производительность различных типов катализаторов на основе платины
За последнее десятилетие многие исследователи сосредоточили свои исследования на разработке электрокатализатора, чтобы повысить его электрокаталитическую активность в метаноле MOR для системы DMFC [37, 38]. Платина (Pt) - это однометаллический катализатор, который проявляет значительно высокую каталитическую активность по отношению к MOR. Однако только чистая Pt в системе DMFC может быть легко отравлена промежуточными частицами, то есть монооксидом углерода (CO), а высокая стоимость катализатора Pt ограничивает его коммерческое применение в качестве электрокатализатора, тем самым снижая кинетическую скорость окисления метанола. в системе DMFC [48,49,50]. Эти три момента являются основными препятствиями и ограничениями использования только Pt в качестве электрокатализатора для DMFC. Однако, чтобы преодолеть эти препятствия, было проведено несколько исследований по синтезу электрокатализаторов из сплавов на основе Pt для достижения лучших электрокаталитических характеристик при меньшем использовании Pt [11, 47, 51, 52]. Обычно средний размер частиц Pt и их морфологию можно определить с помощью сканирующей эмиссионной микрофотографии (SEM) или анализа просвечивающей электронной микрофотографии (TEM), которые являются наиболее распространенными методами в области катализа, которые можно использовать для характеристики физических свойств электрокатализаторов. В таблице 1 показаны средние размеры частиц Pt с различными методами синтеза, свойствами и их характеристиками.
Биметаллический PtRu считается наиболее активным катализатором из-за его бифункционального механизма и лигандных эффектов [48, 53]. PtRu становится интересным каталитическим сплавом, который до сих пор использовался со многими углеродными носителями. Однако токсикологический эффект добавления металлического рутения (Ru) остается неясным [49]. Поэтому были проведены исследования менее дорогих сплавов, в которых Pt смешивается с другими недрагоценными металлами [49,50,51,52, 54,55,56,57], как описано в разделе «Характеристики сплавов на основе Pt».
Характеристики сплавов на основе Pt
Арико и др. [35] обнаружили, что были проведены многочисленные исследования по увеличению каталитической активности Pt-катализаторов в MOR. Во многих исследованиях оптимальное соотношение Pt-Ru было определено как 1:1, а размер частиц в наноразмерном масштабе является идеальным размером для улучшения использования катализатора. Однако Shi et al. [38] определили, что 3:2 было оптимальным соотношением для Pt-Ru в их экспериментах для повышения каталитической активности MOR. Помимо этого, электрокаталитическая активность в отношении активности электроокисления метанола также может быть увеличена, если частицы электрокатализатора PtRu представляют собой наноразмерные частицы в диапазоне 2–4 нм. Paulas et al. [39] согласились с этим утверждением. Как мы знаем, Pt проявляет высокую реакционную способность по отношению к метанольному топливу, что делает металлическую Pt идеальным электрокатализатором для анодного электрода в системе DMFC. Тем не менее, во время MOR катализатора Pt, окись углерода (CO), то есть промежуточные частицы, будет формироваться на поверхности частиц Pt, что, таким образом, отравляет поверхность катализатора [58,59,60,61]. Таким образом, необходимы некоторые усилия для преодоления проблемы, связанной с образованием ядовитых частиц на поверхности частиц Pt, чтобы они не покрывали области активных центров Pt. Обычно бинарные сплавы, такие как PtRu [62,63,64,65,66], PtRh [67,68,69,70,71], PtAu [72,73,74], PtSn [62, 63, 75, 76,77], PtNi [64,65,66,67,78,69], PtCo [70, 71, 78,79,80] и PtFe [81,82,83,84,85], часто используются в качестве комбинаций электрокатализаторов для анодного электрода в системе DMFC. Считается, что добавки этих металлов, таких как рутений (Ru), олово (Sn) и родий (Rh), обеспечивают более высокую каталитическую активность.
Включение никеля (Ni) в катализатор на основе платины дает превосходные характеристики для MOR и DMFC. В последних исследованиях Герреро-Ортега и его сотрудники объясняют, что добавление Ni в подложку Pt-Vulcan способствует значительному увеличению фарадеевского тока во время MOR на один порядок величины, даже несмотря на то, что использование Pt в биметаллическом катализаторе меньше [ 55]. Их экспериментальные результаты также показали, что добавление Ni способствует некоторым структурным и электронным модификациям, которые улучшают характеристики реакции на границе раздела электродов. В другой работе включение Au в сплав Pt усилило электрокаталитическую активность из-за изменения электронной структуры и улучшения электрохимически активной области (ECSA) [47]. В то время как добавление олова (Sn) к сплаву на основе Pt показало увеличение электрокаталитической активности, на которое сильно влияет включение Sn в его систему сплава и окисленные формы, ускоряя реакцию более легко из-за более низкого потенциала окисления [ 56]. Кроме того, добавление кобальта (Co) к сплаву на основе Pt значительно улучшило каталитические свойства катализатора PtCo (1:9) / rGO, который оказался в десять раз выше, чем Pt / rGO [51]. Увеличение плотности тока объясняется более высокой дисперсией наночастиц PtCo по гидрофильной природе носителя rGO, который способствует активации воды и приводит к окислению CO ad на Pt сайтах. Кроме того, согласно бифункциональному механизму Со, он способствует H 2 Активация O, создающая больше ионов -OH и других O 2 -содержащие соединения для окисления СО-промежуточных соединений на сайте Pt [57]. Этот бифункциональный механизм Co может также использоваться для других каталитических переходных металлов в сторону MOR. Механизм каталитического окисления соединений CO до CO 2 в присутствии катализаторов PtCo можно резюмировать следующим образом:
$$ \ mathrm {Pt} + {\ mathrm {CH}} _ 3 \ mathrm {OH} \ to \ mathrm {Pt} \ hbox {-} {\ mathrm {CO}} _ {\ mathrm {ads}} + 4 {\ mathrm {H}} ^ {+} + 4 {\ mathrm {e}} ^ {\ hbox {-}} $$ (4) $$ \ mathrm {Co} + {\ mathrm {H}} _ 2 \ mathrm {O} \ to \ mathrm {Co} {\ left (\ mathrm {OH} \ right)} _ {\ mathrm {ads}} + {\ mathrm {H}} ^ {+} + {\ mathrm {e }} ^ {\ hbox {-}} $$ (5) $$ {\ mathrm {PtCO}} _ {\ mathrm {ads}} + \ mathrm {Co} {\ left (\ mathrm {OH} \ right) } _ {\ mathrm {ads}} / {\ mathrm {CO}} _ 2+ \ mathrm {Pt} + \ mathrm {Co} + {\ mathrm {H}} ^ {+} + {\ mathrm {e}} ^ {\ hbox {-}} $$ (6)Кроме того, Löffler et al. [86] успешно синтезировал PtRu в качестве анодного катализатора для DMFC, с помощью которого был получен наиболее активный электрокатализатор для электроокисления метанола при примерно 50 ат.% Ru. Между тем, Dinh et al. сообщили [87], что PtRu с соотношением PtRu 1:1 имеют более сильные металлические свойства и более высокую электрокаталитическую активность в отношении окисления метанола (MOR). Характеристики связаны с этими двумя основными факторами:(i) максимальная площадь поверхности катализатора и (ii) поверхность катализатора с максимальным количеством центров металлического сплава с атомным соотношением, близким к 1:1. Обе эти группы также показали высокие результаты. Основываясь на бифункциональном механизме, Aricò et al. [58] и Гуденаф и др. [62] предположили, что промежуточные частицы CO, которые образуются на поверхностно-активных центрах Pt, могут окисляться до диоксида углерода (CO 2 ) активными атомами кислорода, образованными на вторичных элементах, например Ru, Sn и Mo, в области нижнего потенциала. В таблице 1 приведены характеристики различных типов катализаторов из сплава Pt, проведенные исследователями для MOR. Согласно бифункциональному механизму [88,89,90], MOR на нанесенных катализаторах из сплава PtRu можно резюмировать в виде следующего уравнения. Pt является более активным катализатором адсорбции метанола, чем Ru. Следовательно, общая реакция на электрокатализаторы PtRu для реакции окисления метанола подчиняется бифункциональному механизму.
$$ \ mathrm {Pt} + {\ mathrm {CH}} _ 3 \ mathrm {OH} \ to \ mathrm {Pt} \ hbox {-} {\ mathrm {CH}} _ 3 \ mathrm {OH} \ mathrm {ads } \ to \ mathrm {Pt} \ hbox {-} {\ mathrm {CO} \ mathrm {H}} _ {\ mathrm {ads}} \ to 3 \ mathrm {H} +3 \ mathrm {e} \ hbox {-} \ to \ mathrm {Pt} \ hbox {-} {\ mathrm {CO}} _ {\ mathrm {ads}} + {\ mathrm {H}} ^ {+} + {\ mathrm {e}} ^ {\ hbox {-}} $$ (7) $$ \ mathrm {Ru} + {\ mathrm {H}} _ 2 \ mathrm {O} \ to \ mathrm {Ru} \ hbox {-} {\ mathrm { OH}} _ {\ mathrm {ads}} + {\ mathrm {H}} ^ {+} + {\ mathrm {e}} ^ {\ hbox {-}} $$ (8) $$ \ mathrm {Pt } \ hbox {-} {\ mathrm {CO} \ mathrm {H}} _ {\ mathrm {ads}} + \ mathrm {Ru} \ hbox {-} {\ mathrm {OH}} _ {\ mathrm {ads }} \ to \ mathrm {Pt} + \ mathrm {Ru} + {\ mathrm {CO}} _ {2+} 2 {\ mathrm {H}} ^ {+} + 2 {\ mathrm {e}} ^ {\ hbox {-}} $$ (9) $$ \ mathrm {Pt} \ hbox {-} {\ mathrm {CO}} _ {\ mathrm {ads}} + \ mathrm {Ru} \ hbox {-} {\ mathrm {OH}} _ {\ mathrm {ads}} \ to \ mathrm {Pt} + \ mathrm {Ru} + {\ mathrm {CO}} _ 2 + {\ mathrm {H}} ^ {+} + { \ mathrm {e}} ^ {\ hbox {-}} $$ (10)Что касается этого бифункционального механизма, метанол сначала диссоциирует и адсорбируется на Pt, а затем разлагается до CO ad и / или формилподобные соединения -CHO ads по реакции дегидрирования (7). В то же время вода диссоциирует на OH ads и адсорбируется на сайтах Ru (8). Затем частицы адсорбируются на сайтах Pt и Ru и объединяются вместе с образованием CO 2 молекула (9) и (10). Реакция между Pt – CO ad и Ru– OHads ведет к CO 2 эволюция, генерирующая обновленные сайты Pt и Ru (реакция 10). Принимая во внимание, что другая работа, выполненная Ewelina Urbanczyk et al. [48] провели реакцию окисления метанола для катализатора PtNi в щелочной среде (1,0 М КОН). Теоретически реакция окисления метанола в щелочной среде выглядит так:
$$ {\ mathrm {CH}} _ 3 \ mathrm {OH} +6 \ mathrm {OH} \ to {\ mathrm {CO}} _ 2 + 5 {\ mathrm {H}} _ 2 \ mathrm {O} +6 { \ mathrm {e}} ^ {\ hbox {-}} $$Реакция начинается на платиновом электроде DMFC с образованием диоксида углерода. Во время этого процесса могут образовываться промежуточные молекулы (CO), которые могут вызвать отравление и дезактивацию активной стороны Pt. Эта молекула CO является продуктом неполного окисления метанола. Неполное окисление метанола приводит к образованию CO в качестве промежуточного продукта (уравнение 11). Поверхность электрокатализатора также может адсорбировать гидроксильные группы (уравнение 6). Наконец, за счет десорбции основного продукта образуется диоксид углерода (13). Второй яд, который может образоваться при окислении метанола, - это метан. В этом случае может протекать следующая реакция (8). Полное окисление промежуточной формы углерода до диоксида углерода в электрохимической реакции выглядит следующим образом:
$$ 3 \ mathrm {Pt} + {\ mathrm {CH}} _ 3 \ mathrm {OH} \ to \ mathrm {Pt} - \ mathrm {COads} +4 {H} ^ {+} + 2 \ mathrm {Pt } + 4e - + {H} ^ 2O $$ (11) $$ \ mathrm {Ni} + {H} _2O \ to \ mathrm {Ni} - \ mathrm {OHads} + {H} ^ {+} + e - $$ (12) $$ \ mathrm {Pt} - {\ mathrm {CO}} _ {\ mathrm {ads}} + \ mathrm {Ni} - \ mathrm {OHads} \ to {\ mathrm {CO}} _2 + {H} ^ {+} + \ mathrm {Pt} + \ mathrm {Ni} + e- $$ (13) $$ \ mathrm {Pt} - {\ mathrm {CH}} _ 3+ \ mathrm {Pt} - H \ to 2 \ \ mathrm {Pt} + {\ mathrm {CH}} _ 4 $$ (14) $$ \ mathrm {Pt} - {\ mathrm {CH}} _ 3+ \ mathrm {Ni} {\ left (\ mathrm {OH} \ right)} _ 2 \ to \ mathrm {Pt} + {\ mathrm {CO}} _ 2+ \ mathrm {Ni} +5 {H} ^ {+} + 5e- $$ (15)В настоящее время исследователи все еще изучают методы легирования для повышения каталитической активности электрокатализаторов на основе Pt путем изготовления тройных и четверных сплавов Pt, таких как PtRuSn [91, 92], PtRuNi [93,94,95], PtRuMo [70, 96 , 97], четвертичный PtRuOsIr [79, 80] и PtRuIrSn [97, 98] из-за их превосходного поведения в MOR и удалении промежуточных частиц (CO), которые образуются на участке поверхности Pt. Но добавление третьего и четвертого металлов в эти тройные и четвертичные катализаторы все еще неизвестно. Более того, существуют некоторые ограничения и проблемы при производстве тройных и четвертичных сплавов. Оптимизация морфологии катализатора и каталитических композиций становится труднодостижимой из-за множества возможных комбинаций металлов и композиций. Однако многие исследования доказали, что добавление третьего и четвертого металлов значительно усиливает каталитическую активность, увеличивает стабильность катализатора и хорошую устойчивость к CO в отношении электроокисления метанола и применения DMFC.
Tsiouvaras et al. [99] провели электрохимические измерения катализаторов PtRuMo / C и обнаружили, что, хотя все тройные катализаторы были более активны в отношении окисления CO и метанола, чем бинарный катализатор, катализатор, обработанный H 2 показали улучшенные характеристики примерно на 15% по сравнению с тройными катализаторами, обработанными He или без обработки. В 2012 году Ху и др. [100] успешно синтезировали превосходный биметаллический (PtNi) электрокатализатор, а именно полые мезопористые наносферы PtNi (HMPNN). Катализатор продемонстрировал выдающиеся каталитические характеристики в MOR со значительно повышенной эффективностью использования Pt из-за уникальной структуры HMPNN и их большой электрохимической площади поверхности. Примерно в 2016 году работа Янга и др. [101] также исследовали реакционную способность синтезированных биметаллических электрокатализаторов PtFe, в которых они обнаружили, что сильное взаимодействие между Pt и металлами железа (Fe) может снижать энергии адсорбции биметаллических НЧ. Они также обнаружили, что биметаллические наночастицы PtFe предпочитают адсорбироваться на графене с одной вакансией через атомы Fe, когда атомы Pt и Fe находятся на поверхности, потому что взаимодействия между атомами Fe и графеном с одиночной вакансией сильнее, чем между атомами Pt и одиночная вакансия графена. На рис. 3 показано положение частиц Pt и Fe, диспергированных на графеновой подложке, как было предложено Янгом и др. [101].
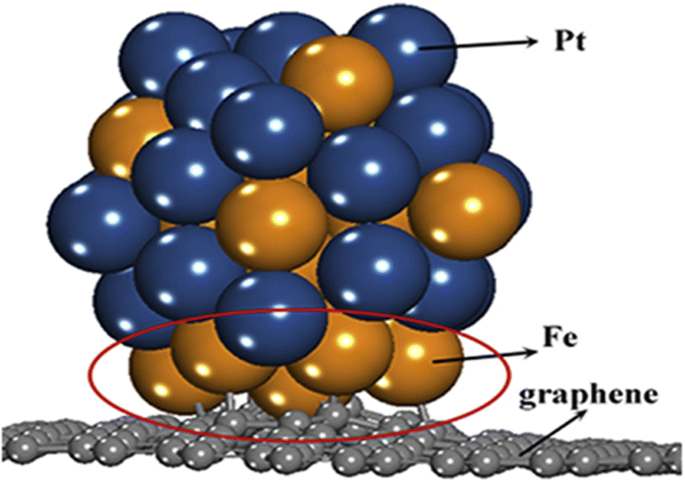
Положение катализатора PtFe на графеновой подложке, проиллюстрированное Yang et al. [101]
Характеристики катализатора на основе платины и карбида переходного металла
Карбид переходного металла (TMC), обладающий высокой механической и химической устойчивостью к коррозии, хорошей стойкостью к кислой среде, долговременной стабильностью и высокой устойчивостью к CO, может действовать как анодный катализатор [88,89,90, 102,103,104]. Кроме того, ТМС также обладают многими преимуществами по сравнению с их исходными металлами в отношении активности, селективности и устойчивости к ядам, например, карбид вольфрама (WC) демонстрирует особые свойства, такие как хорошая электропроводность, устойчивость к кислой среде, низкая стоимость. и толерантность к отравлению CO в процессе электроокисления метанола [88, 105, 106].
Wang et al. [103] сообщили о синтезе с большой площадью поверхности (256 м 2 г −1 ) микросферы карбида вольфрама простым гидротермальным методом. W 2 C был обнаружен в качестве основной фазы в синтезированном образце. В настоящее время исследователи изучают потенциал Pt, нанесенного на WC, в качестве идеального катализатора для DMFC [38, 88, 107, 108]. Christian et al. [106] пришли к выводу, что по отношению к элементам переходных металлов ТМС ведут себя так же, как благородные металлы, такие как Pt, Pd, Rh и Ru, для определенных химических и электрохимических реакций, включая реакцию окисления водорода, монооксида углерода и спирта и восстановления кислорода. [109, 110]. В другом исследовании Liu et al. [107] представили, что карбиды молибдена (Mo-Carbides) могут действовать как промоторы для карбида вольфрама и увеличивать электрокаталитическую активность в DMFC. Однако без включения металлической Pt электрокаталитическая активность чистого WC в отношении MOR для системы DMFC все еще остается низкой. Следовательно, небольшое количество металлической платины, добавленное к компоненту WC, очень удобно для получения преимущества синергетического эффекта между Pt и WC [91, 111, 112]. Между тем, Hassan et al. [109] показали, что обычная примесь (разновидности CO), которая образуется при окислении метанола, имеет сильную энергию связи на поверхности Pt; следовательно, он должен быть окислен, чтобы его можно было удалить с активных центров Pt. Добавление компонента WC в электрокатализатор Pt / WC показало высокую устойчивость к СО для MOR, что указывает на существование синергетических эффектов между металлической Pt и WC в качестве компонента носителя. Исследование также было выполнено другим исследователем с использованием меньшего количества металлической Pt для снижения стоимости Pt при сохранении хороших электрокаталитических характеристик.
В остальном компонент WC более активен для образования метоксигруппы (CH 3 O-), чем чистая Pt [113, 114]. Характеристики CV (Pt:Ru) 4-WC / RGO демонстрируют выдающиеся каталитические характеристики при плотности тока 330,11 мА · мг -1 . Pt по сравнению с другими пятью катализаторами, что указывает на то, что синтезированный электрокатализатор обладает превосходной каталитической активностью в отношении MOR. Кроме того, комбинация Ru и WC на катализаторе Pt увеличивает количество ОН на поверхности и позволяет СО, адсорбированному на поверхности Pt, окисляться при более низких потенциалах [39].
Характеристики катализатора на основе Pt и нитрида переходного металла
Нитрид переходного металла (TMN) является идеальным кандидатом в качестве носителя Pt-катализатора из-за его хорошей электропроводности (металлической), твердости, высокой электрохимической стабильности и коррозионной стойкости в условиях эксплуатации топливных элементов [115,116,117,118]. Сообщалось, что нитриды переходных металлов, такие как CrsN, TiN и VN, на нанесенном Pt катализаторе, показали высокие каталитические характеристики и лучшую стабильность по сравнению с традиционными углеродными носителями [112]. Все переходные металлы могут образовывать нитриды, за исключением металлов второго и третьего ряда групп 8, 9 и 10 (Ru, Os, Rh, Ir, Pd и Pt). Поведение и структурные свойства нитридов переходных металлов можно найти в литературе [92,93,94]. Xiao et al. [112] подготовили Pt-электрокатализатор на подложке из нитрида титана-кобальта, который показал отличные характеристики и стабильность по отношению к реакции восстановления кислорода (ORR). Ti 0.9 Co 0,1 Pt-катализатор на носителе из азота показал малый размер частиц и хорошую дисперсию металлов. Этот приготовленный электрокатализатор также поддерживает электрохимическую площадь поверхности (ECSA) Pt и значительно улучшает сохранение ECSA, с уменьшением только на 35% раннего падения ECSA после 10 000 циклов ADT. Легирование кобальтом значительно увеличило активность и долговечность ORR. Между тем, высокоэффективный и долговечный электрокатализатор в системе DMFC может быть получен с использованием электрокатализатора Pt (Ru) / TiN с большой площадью поверхности, который также продемонстрировал высокую электрохимическую активность в отношении MOR с улучшением каталитической активности на ~ 52% и хорошей стабильностью / долговечностью по сравнению с к рекламе JM-Pt (Ru). Между тем, характеристики DMFC с одним элементом позволили достичь лучшей максимальной плотности мощности на 56% и продемонстрировали выдающуюся электрохимическую стабильность электрокатализатора CSG-Pt (Ru) / TiN [115].
Текущие исследования наночастиц Pt, нанесенных на нанотрубки из нитрида железа титана с полой и пористой структурой и большой площадью поверхности, были проведены Ли и др. [116]. Он показал значительное увеличение электрокаталитической активности по отношению к MOR в кислой среде и имел лучшую долговечность. Причины этих свойств были связаны с их экспериментальными данными, которые подтвердили, что добавление Fe может настраивать электронную структуру атомов Pt, что способствует усиленной активности и стабильности Pt-катализатора для MOR. Между тем, в предыдущей работе, выполненной Xiao et al. [117], Pt / Ti 0,8 Мо 0,2 Катализатор N показал пористую структуру и большую площадь поверхности, а также мелкие и хорошо диспергированные наночастицы Pt. Эта каталитическая система сохранила внутреннюю электрохимическую стабильность наноструктуры TiN и значительно повысила активность и долговечность MOR. Однако имеющаяся в настоящее время информация об электрохимической стабильности нитрида вольфрама (WN) все еще недостаточна [109].
Между тем, MoxN ( x =1 или 2) на титановой подложке проявляет электрохимическую стабильность в кислотном электролите 4,4 M H 2 SO 4 до анодного потенциала +0,67 В (относительно SHE) за 50 повторных циклов [110]. Однако этот электрокатализатор показал поверхностное повреждение, такое как растрескивание и крошение, в областях с высоким катодным (ниже - 0,1 В по сравнению с SHE) и анодным (выше + 0,67 В по сравнению с SHE) из-за катодной и анодной коррозии соответственно. В области высокого анодного потенциала выше +0,67 В (по сравнению с SHE) состав кислорода увеличился из-за образования оксида MoOx, что могло вызвать дезактивацию. Эти результаты показывают, что MoxN реагирует с кислородом, присутствующим в водном электролите, и нестабилен выше +0,67 В (по сравнению с SHE). Mustafha et al. [111] обнаружили, что Pt, загруженная на TiN в качестве носителя, проявляет электроактивность для окисления метанола, с высоким отношением If / Ib, представляющим высокое сопротивление CO на вольтамперограмме, выполненной при скорости сканирования 20 мВ / с в 0,5 M CH 3 OH + 0,5 M H 2 SO 4 как электролит. Бифункциональный эффект между Pt и TiN был назван причиной устойчивости Pt / TiN к СО. Кроме того, Ottakam Thotiyl et al. [91] достигли хороших результатов для катализатора TiN, содержащего Pt, показав очень хорошую устойчивость к CO для электрохимического окисления метанола. Они пришли к выводу, что особые характеристики TiN, которые сделали его пригодным в качестве платиновой основы для MOR в щелочной среде, заключаются в том, что он демонстрирует исключительную стабильность, исключительную коррозионную стойкость, хорошую электронную проводимость и сильную адгезию. Катализаторы на основе TiN обладают преимуществами с точки зрения долговременной стабильности, плотности тока обмена и стабильных токов при низком перенапряжении. В экспериментах использовались 40 мас.% Платины на TiN.
В последние годы Liu et al. [118] successfully synthesized platinum on titanium nickel nitride decorated 3D carbon nanotubes which reduced graphene oxide (TiNiN/CNT-rGO) support by solvothermal process followed by nitriding process. Pt with small particle size is well-dispersed on TiNiN/CNT-rGO support. The 3D shape of CNT-rGO support gives a fast route for charge transfer and mass transfer as well as TiNiN NPs with good synergistic effect and the strong electronic coupling between different domains in TiNiN/CNT-rGO support. Thus, it greatly improved the catalytic activity of this catalyst. In another research, the non-carbon TiN nanotubes-supported Pt catalyst done by Xiao et al. [119] also displayed enhanced catalytic activity and durability toward MOR compared with the commercial Pt/C (E-TEK) catalyst.
Performance of Pt-Based Catalysts with Transition Metal Oxide
Pan et al. [92] reported the synthesis of platinum–antimony-doped tin oxide nanoparticles supported on carbon black (CB) as anode catalysts in DMFC, which exhibited better improvement in catalytic activity toward MOR compared to Pt-SnO2 /C or commercial Pt/C electrocatalyst. The enhancement in activity was attributed to the high electrical conductivity of Sb-doped SnO2 , which induced electronic effects with the Pt catalysts. Another work done by Abida et al. [93]described the preparation of Pt/TiO2 nanotube catalysts for methanol electrooxidation. The TiO2 nanotubes-supported Pt catalyst (Pt/TiO2 nanotubes) exhibited excellent catalytic activity toward MOR and had good CO tolerance. They also reported that the use of hydrogenotitanate nanotubes as a substrate for the Pt catalyst considerably improved the COads oxidation on Pt, but the MOR still occurred at high potential. Then, several years later, Wu et al. [94] synthesized Pt-C/TiO2 with MOR activity 1.6 higher than commercial Pt-C and the stability of Pt-C/TiO2 was also enhanced by 6.7 times compared to Pt-C. The excellent performance of this catalyst was a contribution of mesopores and partially coated carbon support. Zhou et al. [95] prepared hollow mesoporous tungsten trioxide microspheres (HMTTS) using the spray-drying method to yield Pt/HMTTS. The electrocatalyst exhibited excellent electrocatalytic activity and high stability toward MOR than Pt/C and Pt/WO3 electrocatalysts, which may be attributed to the well-ordered Pt particles (with an average size of 5 nm) on the HMTTS surface. Wu et al. [120] used polystyrene spheres as templates to obtain pore-arrayed WO3 (p-WO3 ). The Pt nanoparticles with an approximate size of 3.3 nm dispersed on pore-arrayed WO3 (Pt/p-WO3 ) exhibited high catalytic activity toward MOR.
Ли и др. [121] used Sn-doped TiO2 -modified carbon-supported Pt (Pt/Ti0.9 Sn0.1 О 2 –C) as an electrocatalyst for a DMFC system. The synthesized Pt/Ti0.9 Sn0.1 О 2 –C electrocatalyst revealed high catalytic activity and CO tolerance toward MOR. The enhanced catalyst activity was due to the high content of OH groups on the Ti0.9 Sn0.1 О 2 electrocatalyst sample and the strengthened metals and support interactions. In addition, Lv et al. [122] also reported in their work that the addition of TiO2 could not only facilitate CO removal and hinder CO formation on Pt surface during methanol oxidation, but it can also prevent the agglomeration and corrosion of Pt, which can be concluded from strong metal-supports interaction between TiO2 –C and Pt. Huang et al. [123] revealed that a TiO2 -coated carbon nanotube support for Pt electrocatalysts could be prepared via a one-step synthesis. Hao et al. [124] developed a new catalyst composed of Pt nanoparticles deposited on graphene with MoO3 . These catalysts exhibited high catalytic activity toward MOR and high resistance to CO species. However, the size of MoO3 must be tuned by controlling the metal oxide loading.
The selection of metal oxide such as MnO, RuO, CeO, SnO2 , MgO, and V2 O5 as additional component in electrocatalyst of Pt because of their low cost, good electrochemical properties, and have proton-electron intercalation properties [125]. From the catalytic activity aspect, it can be summarized that the addition of these metal oxides can enhance the electrocatalytic activity of DMFC and other fuel cells. The incorporation of these conducting metal oxides together with Pt catalyst could also facilitate the oxidation process of CO intermediate molecules. Hence, these types of metal have high potential to be used together with platinum as anode electrode.
Carbon support
To improve the utilization of the Pt catalysts, the carbon support is also another useful approach to be used together with Pt. Carbon materials are largely used as catalyst support because of its special properties such as relatively stable in both acid and basic electrolyte, good conductivity, and provide high surface area for dispersion of metal catalyst. It is believed that carbon materials have a strong effect that can influence the electrocatalysts properties such as metal particle size, morphology, metal dispersion, alloyed degree, and stability. Carbon supports can also affect the performance of supported catalysts in fuel cells, such as mass transport and catalyst layer electronic conductivity, electrochemical active area, and metal nanoparticle stability during the operation.
Currently, a great concern of the development in the nanotechnology field, especially carbon nanomaterials synthesis, is to create more stable and active supported catalysts. Support materials of nanoparticles are believed to be the most promising materials for catalytic activity in fuel cells, including the DMFC system. Pt has been traditionally used as nobel catalysts for many fuel cells application [126,127,128]. However, the high cost and low reserve are hindering commercialization of fuel cells and driving researchers to make the utmost of the catalyst. According to this problem, the major effort has been done toward nanoscaling of the catalyst nanoparticles to form more active sites per mass unit. The morphology, structure, and activity of the catalyst, and correspondingly the whole lifetime of a cell, thus strongly depend on the catalyst support [129]. Table 2 shows the preparation, physical properties, performance, and activity of Pt-based supported carbon done by groups of researchers. The details of Pt-based supported carbon will be performed in the following sections:“Graphene Support” to “Carbon Nanocoils”.
Graphene support
Graphene has many extraordinary properties; it exists as a two-dimensional carbon (2-D) form, which is called a crystalline allotrope, one-atom-thick planar flat sheet of sp2 tightly bonded carbon atoms with a thickness of 0.34 nm. Its carbon atoms are packed in a regular atomic-scale chicken wire (hexagonal) pattern [92, 119]. The theoretical specific surface area of graphene is 2630 m 2 g −1 , which is much larger than that of carbon black (typically less than 900 m 2 g −1 ) and carbon nanotubes (100 to 1000 m 2 g −1 ) and similar to that of activated carbon [130]. Graphene has high potential as a metal support [131, 132, 133] [33] due to its high surface area [134] for better catalyst/metal dispersion [135], high electrical conductivity [136], and good thermal properties [137, 138]. Moreover, the functionality of graphene support can be modified by changing it surface structure, and hence contribute to its potential applications, such as in fuel cells, energy storage, electrochemistry, supercapacitors, and batteries [138,139,140,141,142]. Figure 4 illustrates the preparation steps to obtain the graphene nanosheets (GNS), while Fig. 5 shows their TEM images [143]. It can be clearly observed that the thickness of the GO with many typical wrinkles obviously decreases compared to graphite. This can be explained by the presence of the rich oxygen-containing functional groups over the surface of GO [132]. Besides, both resulting GN-900 and GN-900-C contained of a large size of nanosheets structure, but the GN-900-C comprised more transparent than the GN-900.
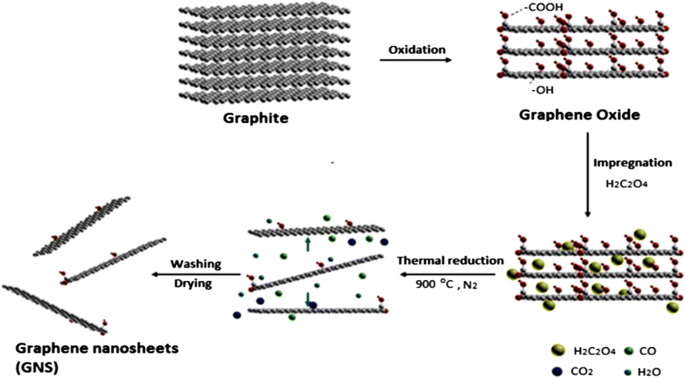
illustration of the preparation of graphite oxide to graphene nanosheets (GNS) by using oxalic acid [143]
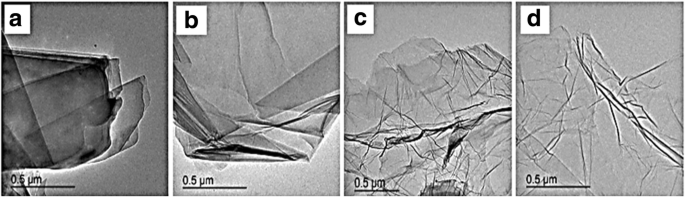
TEM images of graphite (a ), GO (b ), GN-900 (c ), and GN-900-C [143]
The discovery of graphene sheets began around 2000 by mechanical extracting process from 3D graphite source [133]. Graphene can be obtained by several synthesis methods such as hydrothermal [144], chemical reduction [143], chemical vapor deposition, and electrochemical. Ma et al. [145] enhanced the electrocatalytic activity of Pt nanoparticles by supporting the Pt nanoparticles on functionalized graphene for DMFC. Functionalized graphene was prepared by methyl viologen (MV) and Pt/MV–rGO electrocatalyst was synthesized by a facile wet chemical method. They also reported that the higher catalytic activity of Pt/MV–RGO was attributed to the synergetic effect between MV and rGO.
Meanwhile, Zhang et al. [146] modified the graphene support with graphene nanosheets through Hummer’s method, followed by polymerization of aniline (as nitrogen source). The TEM images for Pt/NCL-RGO and Pt/RGO electrocatalysts show that the aggregation between separated graphene sheets was decreased by nitrogen-doped carbon layer (NCL), leading to a better dispersion of the Pt catalyst on the graphene nanosheets support and better electroactivity and stability toward methanol electrooxidation (MOR). Presence of NCL successfully prevented the aggregation of graphene nanosheets as the Pt nanoparticles supporting material.
In 2011, Qiu et al. [135] successfully synthesized nanometer-sized Pt catalyst via sodium borohydride reduction method with an average particle size of only 4.6 nm. These Pt nanoparticles showed an even dispersion of Pt catalyst on graphene oxide support and very high electrocatalytic activity toward MOR by controlling the percent deposition of Pt loaded on the graphene. In another study conducted by Ojani et al. [147], for synthesized Pt-Co/graphene electrocatalyst, it was shown that graphene nanosheets improved the electrocatalytic behavior and long-term stability of the electrode. In addition, the Pt-Co/G/GC electrocatalyst showed great stability toward MOR. The catalytic performance toward MOR can also be improved by using cobalt core–platinum shell nanoparticles supported on surface functionalized graphene [148]. This enhanced catalytic activity could be attributed to the poly (diallyldimethylammonium chloride) (PDDA) that plays a crucial role for dispersion and stabilization of Co@Pt catalyst on graphene support. PDDA-functionalized graphene provided the higher electrochemical active surface area [149, 150]. Huang et al. [138] also studied a PtCo-graphene electrocatalyst with outstanding catalytic performance and high CO tolerance toward the MOR, which far outperformed Pt-graphene and PtCo-MWCNT electrocatalysts with the same ratio of Pt and carbon content. Figure 4 shows the formation of a graphene-PtCo catalyst prepared from a graphite source. Sharma et al. [57] synthesized Pt/reduced graphene oxide (Pt/RGO) electrocatalyst using a microwave-assisted polyol process, which sped up the reduction of GO and formation of Pt nanocrystals. They compared Pt/RGO to a commercial carbon support (Pt/C), which exhibited high CO tolerance, high electrochemically active surface area, and high electrocatalytic activity for the MOR. In a previous study, Zhao et al. [139] reported that the unique 3D-structured Pt/C/graphene aerogel (Pt/C/GA) exhibited greater stability toward MOR with no decrease in electrocatalytic activity. Moreover, the Pt/C/graphene aerogel also exhibited significantly higher stability to scavenge crossover methanol at high potential in an acidic solution compared with the commercial Pt/C electrocatalyst. At the initial catalytic stage, the Pt/C electrocatalyst lost approximately 40% after 1000 CV cycles. In contrast, the Pt/C/graphene aerogel only lost 16% of the initial catalytic activity. After 200 cycles of CV, the current density of Pt/C/graphene aerogel was much higher with a remarkably higher stability than that of Pt/C electrocatalyst. Meanwhile, Yan et al. [151] demonstrated highly active mesoporous graphene-like nanobowls supported Pt catalyst with high surface area of 1091 m 2 g −1 , high pore volume of 2.7 cm 3 g −1 , and average pore diameter of 9.8 nm obtained by applying a template synthesis method. In addition, the Pt/graphene bowls also achieved high performance toward MOR with a current density value of 2075 mA mgPt −1 , which was 2.87 times higher than that of commercial Pt/C (723 mA mgPt −1 ). The onset potential for the Pt/graphene bowls toward methanol electrooxidation was negatively shifted by approximately 160 mV compared with that to the latter and showed CO resistance. Figure 6 shows the proposed schematic for the formation of PtCo catalyst on reduced-GO (rGO) support [51]. It is described that the formation of graphene oxide nanosheets from oxidation of graphite powder leads to the increase in interlayer “d” spacing of stacked graphitic sheets from 0.34 to 0.78 nm due to the presence of various oxygen-containing functional groups. The oxygen-containing functional groups act as anchor sites for the well-dispersed Pt and PtCo nanoparticles on rGO sheets, and used for efficient electrooxidation of methanol.
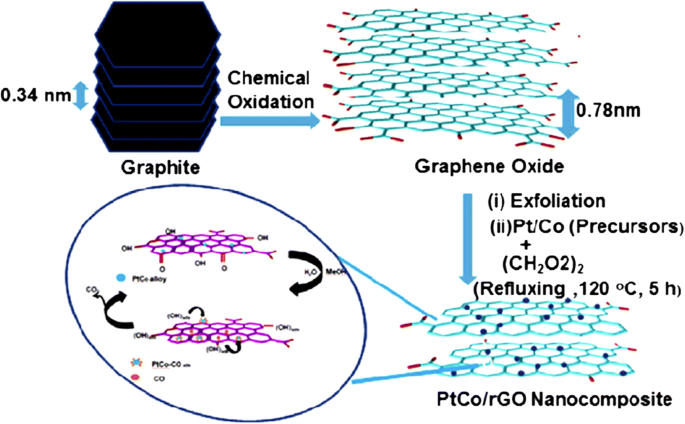
Illustrates the schematic formation of graphene supported Pt-Co catalyst [51]
We can conclude that the reduce graphene oxide (rGO), graphene, modified graphene as supporting material exhibited high electrocatalytic activity toward methanol electrooxidation process. A lot of studies have been reported related to the particle size distribution and size, morphologies, and catalytic activities of Pt and Pt alloys using graphene as supporting material, which showed great improvement in fuel cell performance as mentioned and discussed above. Thus, graphene support can be further studied for better fuel cell performance.
Multiwall Carbon Nanotube and Single-Wall Carbon Nanotube Support
Several years ago, Jha et al. [140] prepared multiwall carbon nanotube (MWCNTs) via chemical vapor deposition using an AB3 alloy hydride catalyst. Platinum-supported MWCNT (Pt/MWCNT) and platinum-ruthenium-supported MWCNT (Pt-Ru/MWCNT) electrocatalysts were prepared by chemical reduction. The performance of these electrodes was studied at different temperatures, and the results demonstrated a very high power density of 39.3 mW cm −2 at a current density of 130 mA cm −2 , which could be attributed to the dispersion and accessibility of the MWCNT support and Pt-Ru in the electrocatalyst mixture for the methanol oxidation reaction. This was also done by other researchers that using different catalyst supported MWCNT for DMFC system [152,153,154,155]. Meanwhile, Wu and Xu [156] compared MWCNT-supported Pt and single-wall carbon nanotube (SWCNT)-supported Pt. Figure 7 shows that the TEM images of Pt catalyst was deposited on MWNT and SWNT electrodes through the electrodeposition technique. The Pt particles in Pt-SWNT (Fig. 7b) looked closer contact with the network of entangled and branched bundles of SWNT support, and the shape is closer to highly exposed sphere. The benefits of the SWCNT support are due to its greater electrochemical surface-active area and easier charge transfer at the electrode/electrolyte interface because of the graphitic crystallinity structure, rich amount of oxygen-containing surface functional groups, and highly mesoporous and unique 3D-structure of SWNT. The electrodeposition technique carried out by them contributed to higher utilization and more uniform dispersion of Pt particles on its support.
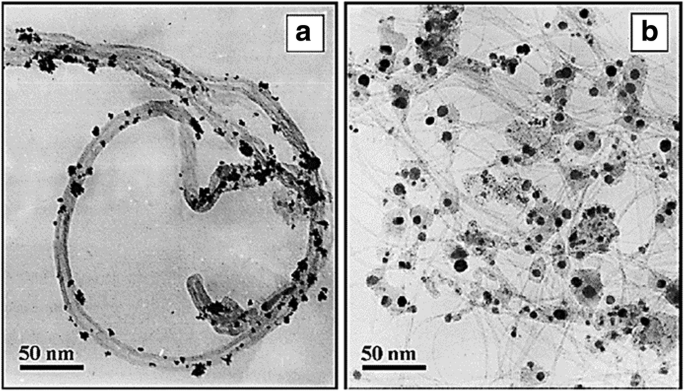
TEM images for the Pt on MWCNT(a ) and SWCNT (b ) [156]
Then, Wang et al. [157] reported the high performance of modified PtAu/MWCNT@TiO2 electrocatalyst prepared via deposition-UV-photoreduction for DMFC, which also exhibited high CO tolerance. Zhao et al. [126] studied 3D flower-like platinum-ruthenium (PtRu) and platinum-ruthenium-nickel (PtRuNi) alloy nanoparticle clusters on MWCNTs prepared via a three-step process, and the best ratios obtained from their experiments for the PtRu and PtRuNi alloys were 8:2 and 8:1:1, respectively. Another group, i.e., Zhao et al. [158], found a higher current density toward MOR and better activity for MWCNT-supported PtWC compared with Pt/C electrocatalyst. These results were attributed to the factors of the synergistic effect between the Pt catalyst and the WC component, high CO tolerance from the bifunctional effect of the Pt catalyst and the WC component, and strong interaction between metals and WC in the electrocatalyst composite.
As a summary, both of MWCNT and SWNT support in terms of structural, surface, and electrochemical properties have their own characteristics as supporting material that remarkably enhanced their performance in catalysis of methanol oxidation process. However, as a comparison, SWCNT possess a high degree of graphitization, highly mesoporous 3D structure, and contain more oxygen-containing functional groups at its surface sites. In relation with these properties, the SWCNT exhibits a higher electrochemically accessible surface area and faster charge transfer rate at the electrode/electrolyte interface.
Carbon Nanotube Support
Wen et al. [144] proposed that carbon nanotubes (CNTs) support could improve fuel cell performance; for example, Pt can be fixed to the inner wall and the outer wall of CNTs and may cause improvement in the electrocatalytic properties of platinum-CNTs. Yoshitake et al. [159] proposed that fuel cells using CNTs as the catalyst support produced larger current densities. The addition of binary or other components to the electrocatalysts for methanol electrooxidation overcomes the problems related to catalyst poisoning caused by CO during the reaction. Therefore, new electrocatalyst carbon supports, such as carbon nanotubes [160, 161], are being actively developed to significantly improve fuel cell performance. Kakati et al. [128] successfully synthesis the PtRu on CNT/SnO2 for anode catalyst DMFC via hydrothermal process. It has been found that the presence of SnO2 provide a high durability property for the catalyst and the presence of SnO2 in the district of Pt could supply oxygen-containing functional groups for the removal of CO intermediate molecules from the Pt surface sites during electrooxidation of methanol. Generally, the decomposition methanol occurs at Pt surface sites; meanwhile, the decomposition of water occurs at SnO2 surface sites to form oxygen-containing species which then react with CO intermediate molecules. However, as support material, the conductivity property of SnO2 still needs to be enhanced. Kakati et al. [128] also proposed the schematic diagram of the formation of PtRu on CNT/SnO2 composite as shows in Fig. 8, and FESEM images of CNT/SnO2 composite support (a and b) and PtRu/SnO2 /CNT composite electrocatalyst (c and d) in Fig. 9.
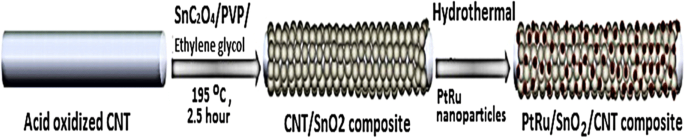
Illustrates the schematic diagram for the formation of PtRu/SnO2/CNT composite [128]
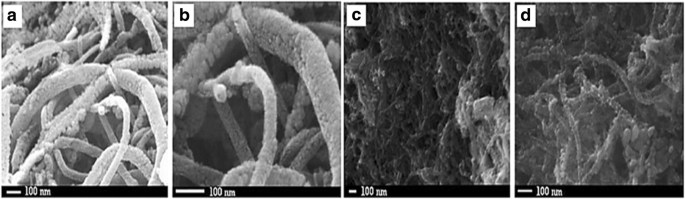
FESEM images of CNT/SnO2 composite support (a , b ) and PtRu/SnO2/CNT composite electrocatalyst (c , d )
Chien et al. [127] proposed that the high catalytic performance of Pt-Ru/CNT for MOR can be attributed to the presence of CNT as the carbon support material with several factors:(i) the as-synthesized Pt-Ru/CNT electrocatalyst owns the ideal nanosized particles and composition to increase catalytic activity, (ii) the presence of functional group on the CNT surface results in high hydrophilicity of CNT, which produces better electrochemical reaction on the electrode area, and (iii) the high electronic conductivity of the CNT support lowers the resistance in MOR. Jeng et al. [150] prepared Pt-Ru/CNT electrocatalyst via a modified polyol with a PtRu composition ratio of 1:1, exhibiting high catalytic activity toward MOR and better performance than that of commercial PtRu/C. Show et al. [162] reported that Pt catalyst with a size of less than 10 nm can be obtained by dispersing the Pt particles on a CNT surface using the in-liquid plasma method, and excellent performance was demonstrated by the electrical power achieving 108 mW cm −2 [162]. The in-liquid plasma method was also used by Matsuda et al. [163] that can applied to obtain nanometer-sized Pt catalyst on support material that remarkably enhanced the fuel cell performance.
To be concluded, high electric conductivity, large surface area, excellent chemical and electrochemical stabilities, quasi one-dimensional structure, and good morphology as the supporting materials are the key factors of carbon nanotubes (CNTs) in enhancing the DMFC performance. In addition, carbon support materials such as CNTs which contribute a large effect on metal distribution and size have also been proven to be an essential to the electrocatalysts to achieve high catalytic activity during methanol oxidation process.
Carbon Nanofiber Support
Steigerwalt et al. [164] reported the successful synthesis of PtRu alloy that was widely dispersed on a graphene carbon nanofiber (CNF) support as an electrocatalyst in DMFC. The catalytic activity was enhanced by ~ 50% relative to that recorded for an unsupported PtRu colloid anode electrocatalyst. Meanwhile, Wang et al. [152] reported that Pt/CNF nanocomposites obtained by the reduction of hexachloroplatinic acid (H2 PtCl6 ) precursor with formic acid (HCOOH) in aqueous solution containing electrospun CNFs at room temperature showed a higher current density than other prepared Pt/CNFs and was approximately 3.5 times greater than that of the E-TEK Pt/C electrocatalyst. Another research carried out by Giorgi et al. [153] described a CNF and bimetallic PtAu electrode with a single layer and both diffusive and catalytic functions using a decreased noble metal amount (approximately five times less) with a consequent large cost reduction. In addition, the bifunctional electrocatalytic properties were also active for the MOR on the PtAu nanoparticle catalysts [154]. Calderón et al. [155] reported PtRu/CNF prepared via reduction using sodium borohydride (NaBH4 ), methanol, and formate ions. This electrocatalyst synthesized by SFM was heat-treated (denoted as SFM TT), which improved its electrocatalytic activity during MOR. Later, Maiyalagan [165] reported that the addition of silicotungstic acid acted as a stabilizer for the PtRu particles on CNT support. The PtRu-supported CNT was prepared by microwave heating of an ethylene glycol (EG) solution of STA, H2 PtCl6 .6H 2 O (as Pt precursor), and RuCl3 .xH2 O (as Ru precursor) with CNF suspended in the solution. The Pt and Ru precursors were loaded on CNF by conventional impregnation method. The results revealed that the PtRu nanoparticles are uniformly dispersed on carbon nanofiber support, with an average particle size of 3.9 nm enhanced the catalytic activity toward methanol electrooxidation. As a conclusion, the carbon nanotubes supporting material with high electronic conductivity and high surface area gives an advantage of better dispersion for the Pt or Pt alloys deposition. The higher the surface area of supporting material can reduce the agglomeration of metal particles on it, thus can produce better catalyst morphology for better fuel cell performance.
Mesoporous Carbon Support
Mesoporous carbon (MPC) support is another ideal candidate as an electrocatalyst support material in DMFC and fuel cell. Generally, mesoporous carbons are divided into two classes based on their structures which are ordered mesoporous carbons (OMCs), with highly ordered pore structure and uniform pore size, nonordered mesoporous carbons with irregular pores. Other than that, OPC can be produced by using high quality of SBA-15 silica and sucrose as carbon source template. To prepare the high quality of SBA-15 SBA-15 sample, triblock copolymer, EO20-PO70EO20 (Pluronic P123, BASF), as the surfactant and tetraethyl orthosilicate (TEOS, 98%, Acros) as the silica source are used, as reported by literature [166,167,168]. The synthesis of MPC starts from synthesis of SBA-15, followed by calcination process.
A well-dispersed and ultralow Pt catalyst (PtFe) supported on ordered mesoporous carbon (OMC) was prepared via a simple route and showed superior catalytic activity. The PtFe alloy with a size range of 3–5 nm was homogeneously dispersed on the CMS with a very high specific surface area of more than 1000 m 2 g −1 [169]. The incorporation of Fe was discussed in the previous section (“Performance of Various Types of Pt-Based Catalysts” section and “Performance of Pt-Based Alloys” section). The high specific surface area of mesoporous carbon support can be produced by carbonization process of a resorcinol-formaldehyde polymer with a cationic polyelectrolyte as a soft template [160]. The performance of Pt/MPC also related to the synthesis/preparation method as done by Kuppan and Selvam. Kuppan and Selvam [167] synthesized four type of Pt/mesoporous carbon by using different reducing agent which are NaBH4 , EG, hydrogen, and paraformaldehyde. From there, the Pt/mesoporous carbon synthesized using paraformaldehyde as reducing agent for showed highest current density. The highest in catalytic was attributed to the use of paraformaldehyde that gives the smallest Pt particle size (4.5 nm), and the highest ECSA (84 m 2 /g) belongs to Pt/mesoporous carbon.
Wang et al. [161] synthesized a Pt@WC/OMC electrocatalyst composite, in which the composite was platinized using a pulsed microwave-assisted polyol technique. The OMC produced in this synthesis exhibited high surface area property. The Pt@WC/OMC electrocatalyst also showed high activity, desirable stability, and CO tolerance toward MOR. In another work done by Zhang et al. [170], the ordered CMS had a unique hierarchical nanostructure (with a 3-D structure) with ordered large mesopores and macropores that facilitated the dispersion of Pt nanoparticles and rapid mass transport during the reactions.
To maximize the use of Pt particles, the support materials should have uniform dispersion, high utilization efficiency, and desirable activity and stability. Moreover, the good supporting materials must be suitable for surface chemistry, high loading of Pt dispersion, and some functional roles. Additionally, based on the previous studies as discussed above, the ordered mesoporous carbons with large pore sizes are highly desirable for fast mass transfer and, thus, enhance the catalytic activity especially in the reaction involve large reactants molecules.
Carbon Black
Carbon black (CB) is one of the commercial carbon support that has been used till now. There are many types of CB such as Vulcan XC-72, Black Pearl 2000, Denka Black, Shawinigan Black, Ketjen EC-300J, etc. [171, 172]. CB is commonly used as a carbon support material for electrocatalysts because it possesses high porosity properties, which make it suitable as a potential support material for the catalyst layer in PEMFCs and DMFCs as reported in provided literatures [173,174,175,176,177,178,179,180]. The comparison of the several carbon black support was reported by Wang et al. [181] who investigated the effect on DMFC performance using several types of carbon black such as Vulcan XC-72R, Ketjen Black EC 300J, and Black Pearls 2000 carbon black as additives/support for the Pt cathode catalyst. From the experiments, the results showed that Ketjen Black EC 300J was the most useful carbon support for increasing the electrochemical surface area and DMFC performance of the cathode catalyst.
Nowadays, CB is commercial carbon support for many fuel cell systems. Generally, it is used for the comparison with new or modified catalyst [125]. The following Table 3 summarizes the commercial carbon black and its properties for fuel cell application. There are so many modifications among carbon support materials and development of new carbon support for enhance fuel cell performance; however, commercial carbon black still is used in many fuel cell applications especially for the comparison with new or modified catalyst.
Carbon Nanocoils
Celorrio et al. [182] proposed carbon nanocoils (CNCs) as a PtRu support in their experiment, indicating that the electrocatalyst performance was strongly dependent on the synthesis method. CNC-supported electrocatalysts showed better electrochemical behavior than E-TEK electrocatalysts, and better electrocatalytic behaviors toward CO and methanol oxidation were achieved using CNC as a support material [182]. Sevilla et al. obtained highly graphitic CNCs from the catalytic graphitization of carbon spherules via the hydrothermal treatment of different saccharides which are sucrose, glucose, and starch [183]. They demonstrated that the high electrocatalytic activity of the CNCs is due to the combination of good electrical conductivity of their graphitic structure and high porosity property, which allows much less diffusional resistance of reactants/products. Two years later, Sevilla et al. [184] reported highly dispersed Pt nanoparticles on graphitic CNCs with diameters in the range of 3.0–3.3 nm and a very fine particle size distribution. The electrocatalyst possessed large active Pt surface area (up to 85 m 2 g −1 Pt), high catalytic activity toward MOR (up to 201 A g −1 Pt), and high resistance against oxidation, which was noticeably greater than that of the Pt/Vulcan electrocatalyst. Celorrio et al. [185] obtained Pt/CNC electrocatalysts via the impregnation method, which showed that a combination of Pt and CNCs facilitated the CO oxidation process.
Conductive Polymer Supports
Choi et al. [186] synthesized PtRu alloy nanoparticles with two types of conducting polymers, i.e., poly(N -vinyl carbazole) and poly(9-(4-vinyl-phenyl)carbazole), as the anode electrodes. Electrochemical and DMFC tests showed that these nanocomposite electrocatalysts were beneficial in a DMFC system, but their catalytic performance was still lower than that of a carbon supported electrode. Thus, they suggested that higher electrical conductivity of the polymer and lower catalyst loss are required in nanocomposite electrodes to achieve better performance in a DMFC. Choi et al. [171] and Kim et al. [172] prepared polyaniline (PANi) as a support material for PtRu catalyst in a DMFC system. PANi is a group of conductive polymers with high electronic conductivity and a methanol oxidation current similar to that of carbon-supported PtRu catalyst. Then, Kim et al. [172] conducted catalytic tests to compare PtRu/PANi support with PtRu/carbon support, showing that the enhanced catalytic activity of PtRu/PANi was due to (i) the high electrical conductivity of the polyaniline support, (ii) the increase of electrochemical surface area of the prepared electrocatalyst, and (iii) the higher ion diffusion behavior. In another study, Amani et al. [74] synthesized PtSn supported by C-PANI as an electrocatalyst with different Pt:Sn atomic ratios using the impregnation method. The PtSn/C-PANI electrocatalyst with a ratio of 30:70 showed outstanding performance in the methanol electrooxidation, and the current density was approximately 40% higher than PtRu/C and 50% higher than Pt/C-PANi. The CO tolerance and stability were improved compared to that of PtRu/C, and the methanol crossover was reduced. Yaldagard et al. [173] studied the electrocatalytic performance of Pt/PANi/WC/C electrocatalyst for methanol electrooxidation (MOR) and oxygen electro-reduction (ORR), and it exhibited higher MOR activity, high CO resistance, and improved stability compared to Pt/C electrocatalyst in the presence of methanol.
Wu et al. [174] presented polypyrrole nanowire networks (PPNNs) as the anodic microporous layers (MPLs) of passive DMFC. In passive DMFC system, the novel MPL achieved a 28.3% increase in the power density from 33.9 to 43.5 mW cm −2 compared with the conventional layer with a similar PtRu (1:1). The high performance was due to the presence of PPNNs, which expressively improved the catalyst utilization and mass transfer of methanol on the anode. Besides, Selvaraj and Alagar [175] prepared Pt-Ru nanoparticle-decorated polypyrrole/multiwalled carbon nanotubes (Ppy/CNT) via the in situ polymerization of Ppy on CNTs containing ammonium peroxydisulphate (NH4 )S2 O8 as an oxidizing agent at the temperature range of 0–5 °C, followed by deposition of Pt particles on PPy-CNT composite films via chemical reduction to produce Pt/PPy-CNT. It was found that the PtRu particles deposited on PPy–CNT composite films exhibited higher catalytic activity and stability toward MOR compared to Pt/PPy-CNT. So far, the investigation on polymer as supporting materials is not much as carbon support materials. From aspect as supporting materials, the performance of polymer support was not good/excellent as carbon support. Further studies are needed in the future for better electrocatalytic activity and DMFC performance.
Problems and Limitations of Using Pt for DMFC Systems
There are two major challenges in the development of new DMFC catalysts:(i) performance, including the catalytic activity, reliability, and durability; and (ii) catalyst cost reduction. Two major problems arise in DMFC when using pure Pt alone as the anode catalysts:(1) slower kinetics oxidation of methanol, even on some state-of-the-art anode catalysts, and methanol crossover through the membrane, which not only lowers cathode performance but also reduces fuel efficiency. To develop successful fuel cell technology, including DMFC technology, new catalysts must be investigated to improve the performance and reduce the cost. Reduction of the catalyst cost remains a major challenge. Currently, platinum is one of the most effective electrocatalysts for DMFC due to its high catalytic activity for methanol oxidation, but because it is a precious metal, platinum usage is challenging and limited [176, 177]. Therefore, many scientists have attempted to find materials that can behave like Pt catalysts. One problem with the MOR in DMFCs is that CO is produced as an intermediate reaction product when using Pt catalyst and has strong binding energy on platinum particles, poisoning the active sites of the platinum surface area [58]. Therefore, CO must be removed by oxidizing it from the Pt surface using another material with high resistance to CO poisoning. For example, Hwu et al. proposed Pt-modified WC catalyst that has remarkable resistance to CO poisoning [178]. On the other hand, they also suggested that CO tolerance originates from the lower CO desorption temperature on pure and Pt-modified WC compared to pure Pt.
There are many solutions that can be applied to reduce the cost of Pt, overcome or minimize the formation of CO species during methanol oxidation, and increase the kinetics of methanol oxidation, such as alloying with other metals or transition metals, the incorporation of metals, metal nitrides, and metal oxides and the use of carbon supports as discussed in this paper. However, to overcome this problem, we need to understand the formation of CO on Pt sites particle, and understanding of the mechanism of the anode reaction in DMFCs. Unfortunately, it has limited amount of mechanistic insight to be studied, because this reactions involve complex mechanism path with many possible intermediate molecules and also competing reaction pathways [179]. For Pt catalytic mechanism, it has been suggested by a direct reaction path. Unfortunately, the use of Pt on other metals has limited mechanistic information available. Figure 10 represents the reaction path for methanol electrooxidation and their possible intermediates molecules formed during the process. Black arrows show direct path, while green arrows show the indirect mechanism for CO2 formation as a final product. In the direct mechanism, the reaction path does not involve a CO intermediate, and CO2 is formed directly from methanol. In contrast, indirect mechanism forming a CO intermediate molecule and subsequently it is oxidized to CO2 продукт. Notably, CO is the most stable molecule of all the intermediates on Pt during MOR. The stability of CO causes it to be a main reason for the extensive CO poisoning problem that is often found on Pt catalyst.
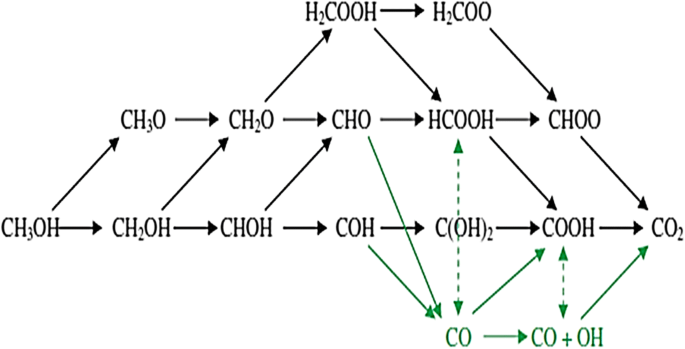
Schematic of the reaction paths and possible intermediates molecules considered in methanol electrooxidation [237]
First step in the mechanism of methanol decomposition reaction on Pt is the activation of methanol molecule. It can take place via hydrogen abstraction from either the carbon or the oxygen atoms. Further step, hydrogen abstraction creates formaldehyde (CH2 O) or hydroxymethylene (CHOH), followed by formyl (CHO) or COH. In the direct mechanism, instead of stripping off the final hydrogen from CHO or COH molecule to CO, a water molecule will release a proton/electron pair and resulting to OH species that can further bind with the carbonaceous species to form dihydroxycarbene (C(OH)2 ) or formic acid (HCOOH). This step is called hydroxyl addition process. The next step is followed by dehydrogenation to form either formate (HCOO) or carboxyl (COOH) molecule, with subsequent dehydrogenation to form CO2 as the final product of reaction. In addition, an alternative direct mechanism involve the stripping of a proton/electron pair from water and addition of the resulting hydroxyl to CH2 O, subsequently to H2 COOH, which then undergoes dehydrogenation to form HCOOH or dioxymethylene (Hs COO). The Hs COO molecule can then undergoes dehydrogenation to HCOO and finally to CO2 . Besides, in the indirect mechanism, CHO or COH species are directly dehydrogenated to CO. Water is dissociated separately on the surface to form OH, and the two surface species react together to form CO2 gas in a way similar to the water-gas-shift reaction [187]. This indirect mechanism occurs because less energy is required to form CO than CO2 . Strong adsorbed CO intermediate form on the Pt surface sites revealed a major problem at the anode site of DMFC. Formation of this intermediate species can cause deactivation Pt catalyst. Furthermore, the rate of kinetic methanol oxidation for DMFC is slower. Therefore, to increase the resistance of Pt catalyst to CO poisoning on the electrodes, Pt alloy or hybrids, such as PtRu, PtSn, PtMO, PtPb, PtFe, PtCo, PtNi, PtRuOs, PtRuMo, PtRuSn, PtRuNi, etc. (as mentioned and discussed in “Performance of various types of Pt-based catalysts” section), are usually employed as electrocatalyst materials on DMFC anodes. Addition/incorporation of these alloys to Pt can prevent the adoption of CO on Pt surface by decreasing the oxidation overpotential of the anode [84].
Conclusion and Prospects
Great progress has been made in recent years in the development and optimization of new catalysts using Pt-based catalysts and carbon and conductive polymer supports for DMFC anode catalyst. Some new carbon materials, such as nano- or mesostructured carbons, have been demonstrated as highly potential catalyst support materials, although their applications face challenges in terms of synthesis, metal loading, and electrode preparation. The combination of platinum as the best metal catalyst for DMFC and an excellent carbon support could produce breakthroughs in the investigation of a new DMFC anode catalyst in the future. Since platinum is an expensive metal, it is necessary to reduce the amount of Pt used in the electrocatalyst. Therefore, this paper presented more than 100 studies on the electrocatalytic activity and performance related to Pt-based electrocatalysts and various carbon and conductive polymer supports. The main problems related to the platinum electrocatalyst, such as carbon monoxide formation during the methanol oxidation reaction and the poor kinetics of methanol oxidation, could be overcome using additional materials and various supports, as reported in the research presented in this paper.
Many studies conducted in the recent years to reduce the loading amount of Pt catalyst and to increase the percentage utilization efficiency, and hence, enhance the electrocatalytic activity of Pt toward the oxygen reduction reaction (ORR) and methanol electrooxidation reaction (MOR), were discussed in this paper. Pt has been alloyed with many transition metals such as Fe, Co, Ni, Ir, Ru, Rh, and Pd, resulting in higher catalytic activity for the DMFC system. The incorporation of these materials also resulted in good dispersion on the carbon and polymer supports, which showed higher performance in the DMFC test compared to the use of Pt metal alone. Various carbon support sources, namely activated carbon (AC), carbon black (CB), multiwall carbon nanotubes (MWCNTs), carbon nanofibers (CNFs), carbon nanotubes (CNTs), graphene, and conductive polymer supports, have been used with Pt-based catalysts to improve their catalytic performance. Additionally, Pt-based alloy catalysts have been designed as hollow mesoporous PtNi, nanowire PtRu, and nanodendritic PtRh, which showed improved electrocatalytic activity and superior electrocatalytic performance. Meanwhile, 3-D Pt/C/graphene aerogel demonstrated enhanced stability toward methanol electrooxidation. The work performed by researchers showed that the electrocatalytic activities of nanoparticles Pt alloy catalysts depend on several factors such as the synthesis method, condition of experiments (such as temperature and pH), alloy composition/ratio, precursors, and thermal treatment. For the future study, it should be extended to the optimization of the geometry and structure of previous studies that revealed active Pt alloys can increase their electrocatalytic activity and stability and the application of support materials for fuel cell applications. For example, current research that have been done by Liu et al. 2017 [188] shows the excellent performance of platinum. From theoretical calculations, it revealed that the main effective sites on platinum single atom electrocatalysts are single-pyridinic-nitrogen-atom-anchored single-platinum-atom centers, which ascribed to the tolerant CO in MOR. They also suggested that carbon black supported used together with Pt single atom is effective in cost, efficient, and durable electrocatalyst for fuel cell application. According to the above study, herein, we can conclude that the modification on structure and morphology of precious metal such as platinum could also remarkably increase the performance of electrocatalyst, but in the same time can reduce the overall cost of fuel cell for commercialization.
To improve the morphologies of Pt and Pt alloys, carbon support material also need further study. Nanoporous metals become an interesting part of catalyst to be studied for fuel cell application. It is determined very suitable for fuel cell catalysts because they possess high surface area, three-dimensional (3D) network structures with adjustable ligament/pore sizes suitable for mass transport, and electron conduction. Around 2017, Li et al. successfully carried out modification on Pt-Pd-Au trimetallic surface as cathode for oxygen reduction reaction [189]. The surface evolution of 3-D Pt-Pd-Au trimetallic greatly enhanced the ORR activity and highly stable as ORR catalyst. The modification of PtNi alloy also done by Li et al. 2016 [190] showed ultrafine jagged platinum nanowire with highly large ECSA that exhibits enhanced mass activity of ~ 50 times higher than state-of-the-art commercial Pt/C catalyst, while Bu et al. 2016 [191] reported highly uniform PtPb/Pt core/shell nanoplate with biaxially strain extremely active, stable for anodic oxidation reactions, and great performance compared to commercial Pt/C in both methanol oxidation reaction (MOR) and ethanol oxidation reaction (EOR). Since the nanostructured platinum becomes an efficient catalyst for fuel cells as well as various industrial chemical reactions. Thus, these modifications on surface of Pt particles electrocatalysts could also to be applied in MOR for future DMFC.
On the other hand, to reduce the consumption of the Pt catalysts, the modification of the carbon support is also another useful way. This not only improves the transport capacity of protons but also reduces the usage of Nafion, which can cut the cost of the fuel cell. Moreover, with regards to the carbon support for the ORR catalysis, the hydrophobic carbon support material is required to allow water (product) to be quickly removed from the catalyst surface sites, and oxygen (reactant) to access the active sites. In contrast, the MOR catalysis requires a certain degree of hydrophilic carbon support. It can be achieved by the modification of the carbon support materials. By combination of modified carbon support materials and development of new carbon support with Pt metal catalyst, it is possible to get an ideal electrocatalysts for direct methanol fuel cell technology. Combination of Pt metal with varied carbon supports with different specific surface areas, structures, pore sizes, electronic properties, and morphologies could be great catalyst to be studied for future DMFC.
Carbon support also influence the overall performance for DMFC. Vulcan XC-72R, which is a commercial carbon support, has a large surface area, appropriate particle size, and good electrical conductivity for good support. However, in the process of depositing metal particle on these support with loading of 40% or more, the particle size of metal increased quickly, which is a disadvantage for DMFC, because a higher metal loading is used to give a better performance. In addition, multiwalled carbon nanotubes (MWCNTs) and carbon nanofibers (CNFs) with relatively smaller surface area, large diameter, and high aspect ratio could be very difficult to deposit a catalyst with high loading metal (40% and more). Therefore, modification of MWCNTs and CNFs support must be done to improve its surface area, surface functional groups, and reduce the wall thickness to achieve outstanding performance for direct methanol fuel cell even though high loading metal catalyst is consumed. As well, a great and important part to be further studied in DMFC system is about the anode and cathode catalyst preparation approaches.
Сокращения
- CB:
-
Carbon black
- CH3 O:
-
methoxy group
- CNC:
-
Carbon nano cage
- CNF:
-
Carbon nano fiber
- CNT:
-
Carbon nano tube
- Co:
-
Cobalt
- Co:
-
Cobalt
- CO:
-
Monoxide molecules
- CO2 :
-
Carbon dioxide
- DMFC:
-
Direct methanol fuel cell
- FC:
-
Fuel cell
- Fe:
-
Iron
- MOR:
-
Methanol oxidation reaction
- MPC:
-
Mesoporous carbon
- MWCNT:
-
Multi wall carbon nanotube
- Ni:
-
Nickel
- OMC:
-
Ordered mesoporous carbon
- ORR:
-
Oxygen reduction reaction
- PANi:
-
Polyaniline
- PEMFC:
-
Proton exchange membrane fuel cell
- Ppy:
-
Polypyrrole
- Pt:
-
Platinum
- Pt/MWCNT:
-
Platinum-supported MWCNT
- Pt-Ru/MWCNT:
-
Platinum-ruthenium-supported MWCNT
- Rh:
-
Rhodium
- Ru:
-
Ruthenium
- Sn:
-
Sternum
- SOFC:
-
Solid oxide fuel cell
- SWCNT:
-
Single-wall carbon nanotube
- TMN:
-
Transition metal nitride
Наноматериалы
- Многофункциональные наночастицы золота для улучшенных диагностических и терапевтических применений:обзор
- Достижения и проблемы флуоресцентных наноматериалов для синтеза и биомедицинских приложений
- Графен и полимерные композиты для суперконденсаторов:обзор
- Изготовление и определение характеристик нового композитного катализатора из углеродного нановолокна Tio2 дл…
- Повышенная производительность нового анодного катализатора PdAu / VGCNF для электроокисления в глицериновом топ…
- Оценка структур графен / WO3 и графен / CeO x как электродов для применения в суперконденсаторах
- Поддержка новых анодных катализаторов для топливных элементов с прямым метанолом:характеристики и характер…
- Биомедицинские приложения для золотых нанокластеров:последние разработки и перспективы на будущее
- Обзор:пористые металлические фильтры и мембраны для разделения масла и воды
- Solvay запускает высокопроизводительную ленту из углеродного волокна для морских нефтегазовых приложений